The snowball hypothesis has properly been questioned and the sharpest questions
to date concern the terrestrial glacial regime, the isotopic evolution of snowball
seawater, and the fate of eukaryotic organisms. These and other questions highlight
the central difficulty with the hypothesis, which is our limited conception
of a snowball event itself. With postulated conditions lying far outside familiar
parameter space, there is danger that the hypothesis we question is a caricature
of a snowball Earth.
Would glaciers flow?
Geological evidence for the existence of dynamic glaciers is incontrovertable.
Many LNGD contain faceted and striated clasts (
Fig. 2b), some far-travelled,
and deformation structures caused by glacial flow (Cahen, 1963; Eisbacher,
1981; Wang et al., 1981; Edwards, 1984; Deynoux, 1985; McMechan, 2000). Associated
sub-glacial pavements (Fig. 2a) are indelibly shaped, scratched, cracked, and
polished by glacial motion (Reusch, 1891; Perry and Roberts, 1968; Deynoux,
1980; Rice and Hofmann, 2000). Are these observations compatible with the cold,
dry atmosphere and limited hydrologic cycle of a snowball Earth (Christie-Blick
et al., 1999; McMechan, 2000)? A major difficulty with this question is that
we do not know which phase of the glacial cycle (Fig. 7) the deposits represent.
A strong bias toward the end-phase is expected because of the temperature trajectory
and because there would have been ample time for ice to thicken even if net
accumulation rates were very low. The magnetic reversal record in the Elatina
(Sohl et al., 1999), however, suggests that a significant time interval is
represented.
Climate models suggest that an ice-albedo runaway might occur very rapidly
(Hyde et al., 2000), freezing the tropical oceans before ice sheets had time
to develop on low-latitude continents (Hoffman et al., 1998b; Pollard and Kasting,
2001). During a snowball event, mean temperatures slowly rise (Fig. 7) in response
to atmospheric CO
2 and this will cause an exponential increase in saturation
vapour pressures. Moisture sources include subaerial volcanic outgassing, sublimation
of tropical sea ice (Warren et al., 2002), and evaporation of transient melt
ponds (Walker, 2001), leads and polynyas within the tropical ice pack (Kirschvink,
1992). Given large seasonal and diurnal fluctuations in surface temperature
(Walker, 2001), water vapor would be advected to topographic highs on summer
afternoons and moisture would condense there forming glaciers (Walker, 2001).
Due to the weak hydrologic cycle, snow accumulation rates would be extremely
low, but a positive feedback would develop between surface elevation and snow
accumulation if glaciers thickened. Over sufficient time, these glaciers must
become wet-based and flow, possibly in localized ice streams or as episodic
ice surges (Bentley, 1987; MacAyeal, 1993; Condon et al., 2002). The observed
zonal distribution of LNGD (
Fig. 1) might broadly reflect the zonal pattern
of precipitation minus ablation on the snowball Earth. On the regional scale,
the importance of topography, particularly coastal topography, in capturing
the limited precipitation is not inconsistent with the common observation that
thicker glacial deposits occur in tectonically more active areas and times
(Young, 1995). They commonly overlie pre-glacial strata with angular unconformity,
consistent with a prolonged hiatus (with ongoing block rotation) between the
ice-albedo runaway and the onset of glacigenic sedimentation (Fig. 7). However,
LNGD are missing or very thin beneath cap carbonates in many areas (Wright
et al., 1978; Preiss, 1985; Aitken, 1991; Hoffmann and Prave, 1996), or aeolian
sandstone overlies permafrost regolith in place of LNGD (Deynoux et al., 1989;
Moussine-Pouchkine and Bertrand-Sarfati, 1997; Williams, 1998). As lag deposits
or other evidence of erosion of LNGD before cap-carbonate deposition is generally
lacking, the observed stratigraphic relations indicate that ablation exceeded
precipitation through the glacial cycle in many areas. Where LNGD are absent,
without evidence for removal by erosion, or where their position is occupied
by aeolianite (Deynoux et al., 1989; Moussine-Pouchkine and Bertrand-Sarfati,
1997; Williams, 1998; Fig. 5a), ablation evidently exceeded precipitation over
the full glacial cycle.
Would seawater change?
The elemental and isotopic composition of seawater should change in a snowball
event. Decimated organic productivity and hydrothermal dominance should cause
δ
13C and
87Sr/
86Sr, respectively, to fall (Kump, 1991; Jacobsen and Kaufman,
1999). The trajectories of the changes depend on the C and Sr residence times,
and are sensitive to buffering by carbonate dissolution (Jacobsen and Kaufman,
1999) in response to submarine volcanic exhalations of CO2 and other acids.
Consequently, few δ
13C or
87Sr/
86Sr values have been reported for the glacial
ocean because primary syn-glacial carbonates are rare. Kennedy et al. (2001b)
report positive δ
13C values from purported examples in Australia, Namibia and
California, whereas Xiao et al. (2001) find that thin calcareous crusts in
the Tereeken glacials (
Table 1) of NW China have δ
13C close to -5‰.
Cap carbonates have been substituted as proxies for seawater
87Sr/
86Sr during
snowball events (Jacobsen and Kaufman, 1999; Kennedy et al., 2001b), justified
by the Sr residence time of ~3.5 myr in the present ocean. Neoproterozoic Sr
isotope data are not very reliable because strontium is a mobile trace element
and most carbonates of that age are totally recrystallized (Derry et al., 1992).
Nevertheless, the “least altered”
87Sr/
86Sr ratios in cap limestones
(dolomites have very low Sr concentrations) are not significantly different
from pre-glacial values (Jacobsen and Kaufman, 1999; Kennedy et al., 2001b).
This may be interpreted in two ways. Either the
87Sr/
86Sr ratio of seawater
changed little because the glaciation was short-lived (Jacobsen and Kaufman,
1999), contrary to paleomagnetic (Sohl et al., 1999) and subsidence (Hoffman
et al., 1998a) data, or else the ratio was buffered by carbonate dissolution
during the hypothesized snowball event and enhanced weathering in the greenhouse
aftermath (Hoffman et al., 1998b). If atmospheric pCO
2 rose from a pre-glacial
value of 0.0003 bar to 0.12 bar over the course of a snowball event (Caldeira
and Kasting, 1992), then the Ca ion concentration of seawater would have to
increase five-fold
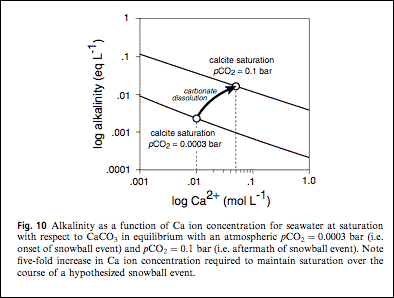
simply to maintain carbonate saturation (Fig. 10). This
enormous Ca input would be accompanied by a flux of isotopically conservative
Sr from carbonate dissolution and radiogenic Sr from silicate weathering. These
inputs might greatly reduce or even eliminate the predicted lowering of
87Sr/
86Sr
during a snowball event of any given duration (Jacobsen and Kaufman, 1999).
The large magnitude of the resulting seawater Sr reservoir, plus the rapid
weathering of carbonates and young volcanics, would damp any increase in seawater
87Sr/
86Sr due to silicate weathering, consistent with available data (Jacobsen
and Kaufman, 1999; Kennedy et al., 2001b). Contrary to Kennedy et al. (2001b),
the transient weathering flux is not limited to the timescale of the sea-level
rise (<104 years) but continued long after as indicated by the negative
δ
13C anomaly (Fig. 9) which encompasses the entire transgressive-regressive
post-glacial sequence. Accordingly,
87Sr/
86Sr ratios in cap carbonates are
broadly similar to pre-glacial values but should rise thereafter due to silicate
weathering, as is observed (Shields et al., 1997).
Would eukoryotes survive?
Few biologists doubt that prokaryotic organisms could survive snowball events
(Priscu et al., 1998; Gaidos et al., 1999; Vincent and Howard-Williams, 2000;
Thomas and Dieckmann, 2002). Extant clades of eukaryotic algae are known from
strata around 1.0 Ga (German, 1990; Butterfield et al., 1990, 1994; Butterfield
and Rainbird, 1998; Butterfield, 2000) and they must have prevailed as well.
A marked decline in eukaryotic and total microfloral diversity encompasses
the late Neoproterozoic glacial events (Vidal and Knoll, 1982; Schopf, 1991;
Knoll, 1994; Vidal and Moczydlowska-Vidal, 1997), and it is not until well
after the Elatina/Nantuo glaciation (
Table 1) that an abrupt increase in abundance,
size, morphological complexity, and taxonomic diversity of acanthomorph acritarchs
is observed (Zhang et al., 1998; Grey, 1998; Walter et al., 2000). The enigmatic,
soft-bodied, Ediacara macrofossils—arguably dominated by cnidarian-grade
metazoans—appears world-wide by 575-555 Ma (Jenkins, 1992; Fedonkin,
1992; Seilacher, 1992; Martin et al., 2000). In northwestern Canada, simple
Ediacara-type fossils with radial symmetry occur in the immediate post-glacial
sequences of both the Rapitan (Hofmann et al., 1990) and Ice Brook (Narbonne
and Aitken, 1990; Narbonne, 1994) glaciations (
Table 1). Many molecular-based
age estimates of the last common ancestor of bilaterians predate the hypothesized
snowball events (Runnegar, 2000), but there are good reasons to take these
estimates lightly (Peterson and Takacs, 2001).
Assuming snowball events occurred, how did eukaryotic plankton survive? In
what refugia did early metazoans, if they existed, ride out the climate shocks
entering and exiting the hypothesized snowball events? Did their prolonged
isolation contribute ultimately to evolutionary diversification? Potential
refugia include brine channels in juvenile sea ice (Thomas and Dieckmann, 2002),
leads and lanes in the tropical ice pack (Kirschvink, 1992; Lemke, 2001), tidal
cracks along ice-grounding lines (Gaidos et al., 1999), transient meltwater
ponds (Walker, 2001), thin equatorial sea ice (McKay, 2000), and hot springs
around volcanic islands (Hoffman and Schrag, 2000). Snowball seawater would
be laden with nutrients due to hydrothermal activity and limited organic productivity
(Kirschvink, 2000). Algal “blooms’ and enhanced organic burial
associated with rapid sedimentation when snowball oceans were uncorked would
have released oxygen to the atmosphere (Kirschvink et al., 2000; Walter et
al., 2000). This is supported by evidence of increased environmental oxygenation
roughly coincident with both the Paleoproterozoic (Prasad and Roscoe, 1996;
Rye and Holland, 1998; Farquhar et al., 2000; Murakami et al., 2001) and Neoproterozoic
(Des Marais et al., 1992; Logan et al., 1995; Canfield and Teske, 1996) snowball
eras (Fig 12). The isotopic signal of such organic-burial events might be muted
if they were accompanied by a high carbonate burial flux, driven by weathering
as we have argued. The rise of the Ediacaran biota has long been attributed
to a rise in molecular oxygen (Runnegar, 1982; Knoll and Carroll, 1999), but
it is not clear what stabilized pO
2 at higher levels following the perturbations.